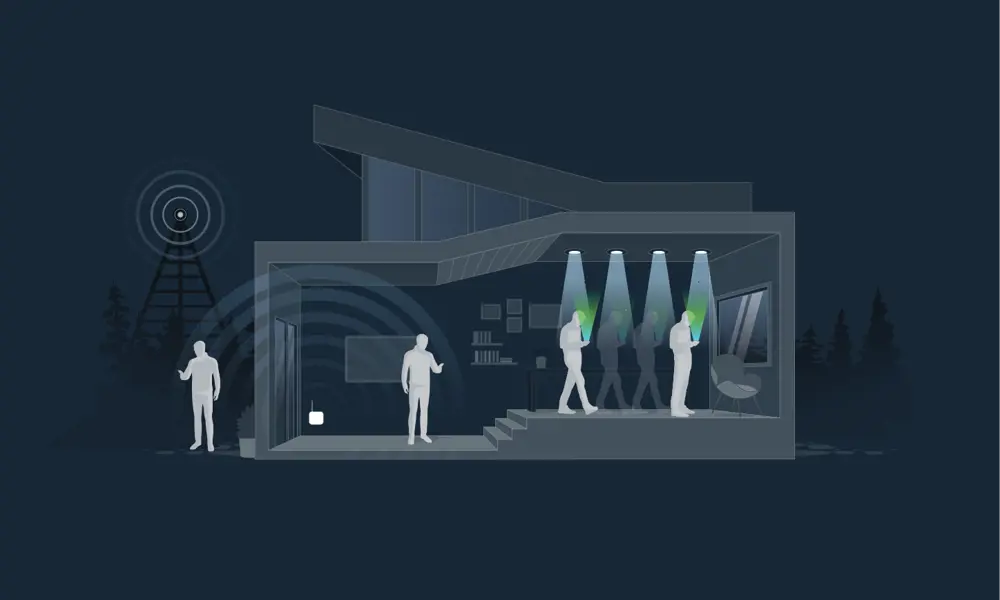
Communication at the speed of light
History has many ‘what if’ scenarios and the development of radio communications may have inadvertently prevented another technological breakthrough. In 1880, Alexander Graham Bell developed a photophone that used a beam of light to transmit messages across the visible light communications (VLC) spectrum. However, subsequent advances in radio wave technology eclipsed Bell’s work and the 20th century was consequently dominated by communication across the radio frequency (RF) spectrum.
Fast forward to the present day and another VLC breakthrough is imminent. Engineers have been looking beyond RF wireless cellular communications and Wi-Fi to satisfy our spiralling requirements for high-speed data transmission. Unlocking the potential of high-frequency, short-wavelength VLC bands has become crucially important as global data traffic continues to rise, with light fidelity’s (Li-Fi) potential currently being tested in proof-of-concept trials around the world.
Rather like the triangulation of mobile devices, where a series of cellular base stations and localised micro-cells offer connectivity at any given moment, Li-Fi data is distributed through coordinated transmissions from various light sources
LEDs make the concept of a wireless data network provided by existing light sources possible. All digital data is coded in binary form – zeroes and ones – which also correspond to the status achievable by a light element. By turning a light on and off within a few dozen nanoseconds, it is possible to transmit huge amounts of data to nearby objects. The human eye is unable to identify any fluctuation in light levels, but any device equipped with a compatible photodiode receiver can detect the presence or absence of individual light photons.
Rather like the triangulation of mobile devices, where a series of cellular base stations and localised micro-cells offer connectivity at any given moment, Li-Fi data is distributed through coordinated transmissions from various light sources. Dense networks with multiple cell sites improve achievable data rates, while creating a wider network of coverage. For instance, a table lamp and spotlight at opposite sides of a room could each distribute their own arcs of light, with algorithms seamlessly handing over from one device to another as the data receiver, such as a smartphone, moves between them. The frequencies used to transmit Li-Fi data are almost completely resistant to interference from sunlight, so low-energy streetlamps could provide a stable connection for people walking down a pavement, even on a bright day.
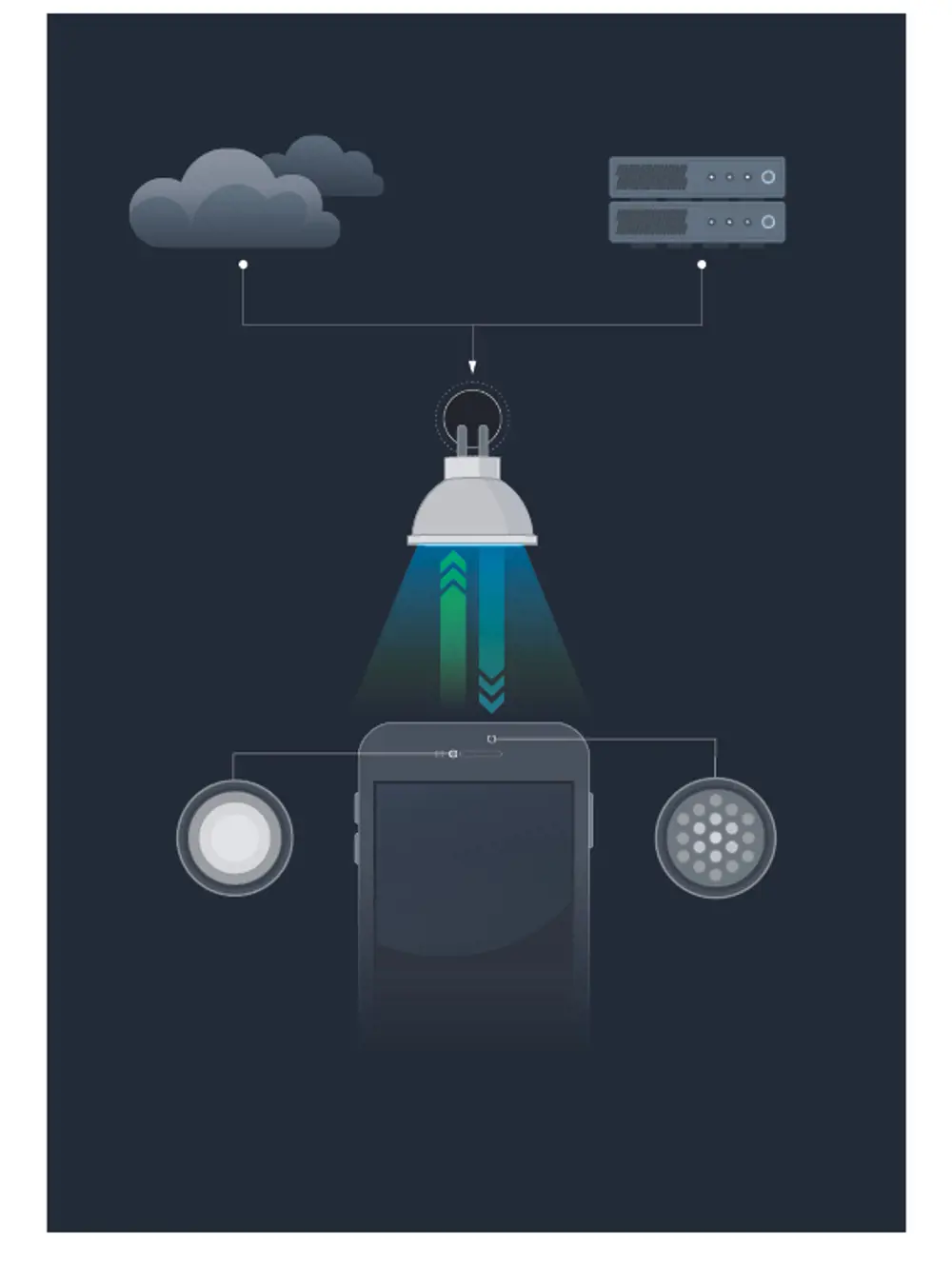
The key building blocks of a bi-directional Li-Fi communication link consist of an infrared transmitter and a photodetector, integrated into a mobile device. A lightbulb at the other end serves as a wireless access point and also has an integrated photodetector to receive data. The lightbulb is connected to a data server or cloud architecture, which provides a connection to the internet © pureLiFi
Light benefits
Li-Fi offers several advantages, as Professor Harald Haas from the University of Edinburgh explains: “It’s secure, simply because light doesn’t go through walls. Somebody on the street would find it hard to build an optical system to intercept that light … whereas with Wi-Fi, data interception can be achieved from a safe distance. That makes Li-Fi ideal for offices, banks and insurance companies, where data sensitivity is very important.” He cites the example of manufacturing facilities, where terabytes of data could be transmitted from robots to the cloud: “nobody outside the halls could read the signal and write down the data sets, because manufacturing facilities rarely have windows.”
Li-Fi also enables precise location services, which could be used to build a histogram of movement that artificial intelligence and machine learning could use to determine typical user behaviour. For example, says Professor Haas: “if someone is meant to be in a meeting room every Friday at 10.00am but there’s one Friday where they are in a secure server room, the system could flag an anomaly. You might view that as ‘Big Brother is watching’, but from a cybersecurity perspective, the enemy is often inside the system.”
Data transmitted across light-based communications links also avoids interference with electrical equipment, which means Li-Fi is suitable for use in environments where RF transmissions are restricted. For example, an uninterrupted data flow could be provided through the reading lights on aeroplanes or through existing ceiling lighting in hospitals.
Li-Fi operates well in low light conditions, as Professor Haas explains: “there’s a minimum amount of light required depending on the respective photo-receiver technology. As a consequence, one of the existing challenges in the design of Li-Fi systems is the receiver. What’s important is that a signal is received optimally at the receiver. A typical photodetector device takes the fluctuating light photons, which carry the information, and converts them into an alternating current. That needs to be converted into voltage signals using a transimpedance amplifier – an operational amplifier that takes the alternating current and turns it into an alternating voltage signal that can be processed by analogue-to-digital converters.” However, there have been significant advances in the research of new detectors and optical components recently. Several photodetector technologies are in development, including highly sensitive single photon avalanche diodes that count individual photons and need almost no light input into the receiver and no transimpedence amplifier. However, this particular detector can be easily saturated by ambient light. Other photodetector technologies are characterised by the trade-off between bandwidth and sensitivity. Generally, a larger detector area can collect more photons and achieve higher receiver power, but this also means that the detector has a high capacity that limits the bandwidth. To overcome this limitation, optical concentrators can be used in conjunction with small area detectors. However, optical concentrators reduce the bandwidth of the system, which is an issue to overcome. Therefore, dealing with low light levels is still an ongoing area of research and development. Sunlight or bright light interference can be less of an issue: using appropriate optical and electrical filtering techniques in combination with appropriate digital data encoding techniques, the impact of strong sunlight interference can be reduced to less than 5% degradation of achievable data rates.
LEDS
🚨 Turning LEDs into high-speed data transmitters
Any LED light, including status lights on microwaves or fridges, torches, car headlights or streetlamps, can be turned into a high-speed data transmitter, whether organic, micro, different colour or single device white LEDs.
Professor Haas’s research team has used a single colour LED purchased for less than 10 pence to transfer more than 4 Gbps (billions of bits per second) of data – faster than the best speed achievable with the latest Wi-Fi systems, which top out at around 867 Mbps (millions of bits per second).
The achievable data rate performance of Li-Fi significantly depends on the light source and the detector. The bandwidth of each technology is different and ranges from 2 MHz (megahertz) in the case of phosphor-coated white LEDs to 1 GHz (gigahertz) in the case of micro LEDs. Current low-cost commercial white light LEDs are composed of a blue LED chip and a layer of a phosphorous material that converts some of the blue photons to yellow photons; these mix with the blue photons to create white light. Unfortunately, the phosphorous coating reduces the bandwidth of the device. As a result, the maximum data rate is in the region of tens of Mbps. This can be eliminated by using different colour LEDs such as red, green or blue LEDs, which has two effects: there is no need to use the coating as light can be mixed into any colour including white, and as a consequence the bandwidth is significantly increased by one or two orders of magnitude; and each colour could carry independent data and as a result, the data rate linearly scales with the number of colours. Therefore, multicolour LEDs can achieve data rates of 50 Gbps (assuming approximately 12 colours) and higher. Lastly, the automotive sector uses white light sources that use laser diodes to produce white light that is harmless to eyes. It is conceivable that these lighting devices will be introduced to the consumer lighting market within the next few years and, as laser diodes have much higher bandwidth than LEDs, this would mean that future lightbulbs could transmit data at speeds in the Tbps (terabytes per second) region.
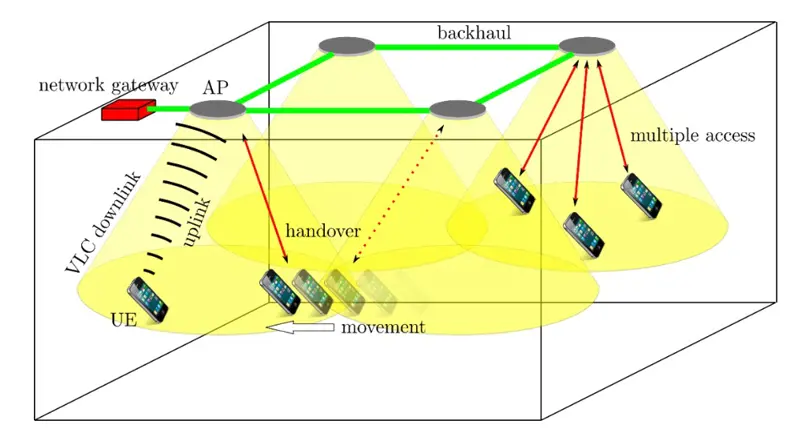
This diagram shows how ordinary LED lightbulbs become Li-Fi access points. They are connected via a high-speed data backhaul, such as power-over-ethernet (PoE), which means that power and data is supplied simultaneously by one connection that can provide up to 60 watts. As lamps are not connected to the main power supply, entire buildings can be fully equipped with PoE lighting. In the case of retrofitting, a different technology, such as powerline communication, would be more advantageous to avoid new cabling. This uses the main power supply to distribute data
Li-Fi in reality
Li-Fi is currently being used in more than 130 real-world deployments in over 23 countries, including one notable example at a high school in Ayr. As the first school in Scotland to adopt Li-Fi technology, Kyle Academy has a classroom equipped with eight ceiling-mounted LED lights that provide speeds in excess of 340 Mbps (millions of bits per second). As well as providing high-speed data access for pupils and teachers within that particular room, the introduction of Li-Fi has improved Wi-Fi speeds in neighbouring rooms, because fewer people were using the available RF bandwidth. This means that losses caused by data collisions (when devices try to get access to the Wi-Fi channel) have been reduced, resulting in a significant efficiency improvement in Wi-Fi. In the past few years, Wi-Fi has worked as a ‘pressure relief valve’ for 4G data traffic through data offload. This has aggravated the congestion issue in Wi-Fi in crowded places, such as schools. The school trial has shown that Li-Fi can work very effectively as a powerful ‘pressure relief valve’ for data offload from Wi-Fi.
In contrast to point-to-point optical wireless communication, which uses VLC, a Li-Fi system is defined as a fully networked, multiuser, bi-directional cellular communications system. It includes multiple access points that form cellular networks, which are constructed of many ultra-small communication cells, to provide high-data-density wireless communication services to multiple mobile users simultaneously. The Li-Fi network supports handover when users move across different light coverage regions.
The school trial has shown that Li-Fi can work very effectively as a powerful ‘pressure relief valve’ for data offload from Wi-Fi
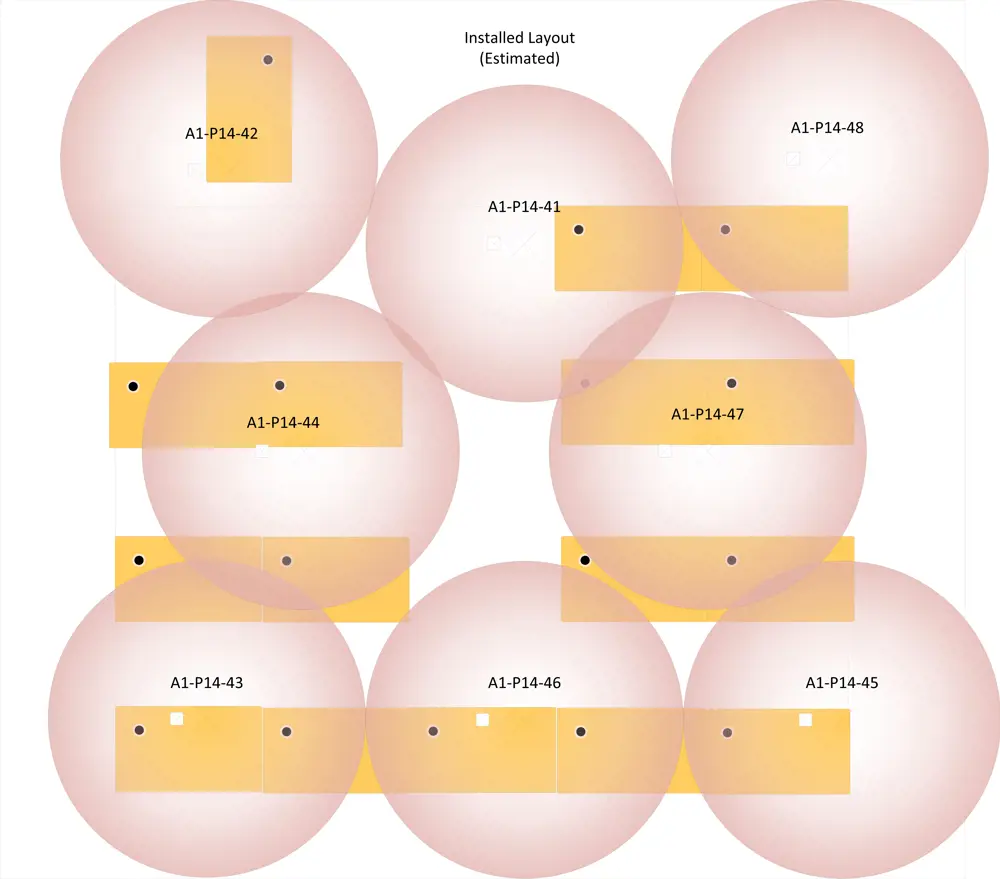
This diagram shows a real-world Li-Fi installation in a school classroom. Usually, there is at most one Wi-Fi router per room, but Li-Fi makes it possible to have almost one wireless router per desk yielding a much higher data density. The Li-Fi network installed here consists of eight Li-Fi access points mounted in the ceiling (the circles) to provide coverage to the students’ and teacher’s desks (the yellow boxes)
In addition, connections between access points and network gateways are essential to enable cooperation and establish connection to the wider communication network. The Li-Fi downlink piggybacks on existing LED lighting systems, which are becoming increasingly popular. The systems also comprise of an optical uplink – the link between the mobile terminal and the access point. Since using visible light in the uplink may cause distraction to the mobile user, the use of the infrared spectrum is deemed most appropriate for this link direction as there is no interference between uplink and downlink and simultaneous communication links can be established.
Since the lighting system now has two primary functions – lighting and mobile connectivity – there is a natural trade-off when it comes to installation. In some instances, the communication requirements are different from the lighting requirements, which would demand a different lighting layout. However, research has shown that as lighting typically ‘follows’ users, the optimum lighting layout mostly leads to almost optimum communication performance in typical indoor situations. Also, it is important to be able to communicate while the lights are off, so the communication performance must be largely agnostic to the dimming levels. Professor Haas’s team’s work has shown that this can be achieved by designing new digital modulation techniques such as enhanced unipolar orthogonal frequency division multiplexing (OFDM). This can significantly reduce the emission of photons that are not used for communication purposes by superimposing multiple parallel data streams in time and frequency in such a way that the constant light output, which primarily serves to illuminate, can be reduced to close to zero. Moreover, OFDM divides the entire bandwidth into multiple subchannels that can be grouped together to form a data stream. This provides multiple advantages. One of the most important ones is that each subchannel can be treated independently. Typically, there are subchannels that offer good conditions and others are highly attenuated, especially at the upper region of the bandwidth. With OFDM it is easily possible to vary the transmission rate per subchannel to adjust to the varying channel conditions and always ensure that the bandwidth is used optimally. This is an important requirement for mobile use cases where the channel constantly and randomly changes. If communication is required when the lights are off completely, such as in an aircraft cabin at night, the integration of infrared light sources alongside visible light LEDs provides a straightforward solution.
Adoption of existing optical communication technology for Li-Fi is possible to a certain extent. For example, remote controls that use infrared light for low level and low data rate control are based on pulsed modulation techniques, which can be limited in high-speed mobile communication environments. Reflections from walls and objects cause interference and line-of-sight cannot be guaranteed at all times. For example, with a mobile phone that constantly changes orientation, the Li-Fi system must be designed to be robust to such random changes. As a consequence, it demands different optical communication technologies than used in applications where there is always line-of-sight between the transmitter and the receiver such as TV remote controls, which directly point to the TV, or outdoor long-range optical links installed on roofs or masts, or ground-to-satellite optical communication systems, which use tightly focused lasers.
The future of Li-Fi
According to Cisco Vision, by 2020 96% of all mobile data traffic consumption will be indoors. Li-Fi’s potential for internal data transmission represents the greatest driver behind the technology. The 2.4 GHz (gigahertz) industrial, scientific and medical (ISM) band (used for RF transmissions) had become so congested across its 150 MHz (megahertz) of bandwidth that new bandwidth in the 5 GHz region has been unlocked to provide additional bandwidth of about 500 MHz. Even then, the plethora of electromechanical devices attempting to use this bandwidth cause data loss, interference and slow transfer speeds, which are incompatible with the always-on, ultra-fast connectivity required to transmit the Internet of Things’ exponentially increasing data volumes.
According to Cisco Vision, by 2020 96% of all mobile data traffic consumption will be indoors. Li-Fi’s potential for internal data transmission represents the greatest driver behind the technology
Professor Haas believes that Li-Fi will go hand-in-hand with the Internet of Things, where formerly passive devices perform specific tasks using internet connectivity. From self-replenishing fridges and app-connected bathroom scales through to autonomous vehicles and remote surgical procedures, an estimated 125 billion devices will require online connectivity by 2030. The RF wavelengths currently being used are considered slow and congested to adequately service these vast amounts of data and also provide the required quality of service, such as low-latency requirements for mission-critical applications. Even the forthcoming 5G infrastructure may be insufficient. By contrast, Li-Fi has around 780 THz (terahertz) of bandwidth ready to be used, across a light spectrum 2,600 times larger than the entire RF spectrum.
From self-replenishing fridges and app-connected bathroom scales through to autonomous vehicles and remote surgical procedures, an estimated 125 billion devices will require online connectivity by 2030
It seems that Li-Fi is inevitable given the continued growth of mobile data transmission. However, this technology will only move into the mainstream on a larger scale once original equipment manufacturers start adopting Li-Fi technology in their devices, in the same way as they have previously introduced 4G and Bluetooth. Professor Haas views Li-Fi as complementary to 5G, rather than a replacement: “If you have your device in your pocket, you’d use Wi-Fi for message notifications, because Li-Fi cannot penetrate fabrics very well. It’s a question of where the technology is best used. If the device is taken out of the pocket and has a good connection, you would use Li-Fi. If you are in areas where there is no light coverage, or the lights are completely off, obviously you would have a seamless handover to Wi-Fi. Li-Fi is complementary to Wi-Fi, in the same way Bluetooth and 4G work alongside Wi-Fi now.”
Professor Haas believes the Li-Fi revolution could be imminent: “Given that we are on the pathway to developing a standard in microchips that allow integration and scalability, I reckon people will be able to buy Li-Fi-enabled products within the next two to three years.” That is worth remembering next time you turn on a lamp or watch a standby light glowing on an electrical device.
***
This article has been adapted from "Communication at the speed of light", which originally appeared in the print edition of Ingenia 78 (March 2019).
Contributors
Professor Harald Haas is Chair of Mobile Communications at the University of Edinburgh and Co-Founder and Chief Scientific Officer of pureLiFi. He is also Director of the LiFi Research and Development Centre at the University of Edinburgh. He first introduced and coined Li-Fi in a TED Global talk in 2011. Subsequently, Li-Fi was listed among the 50 best inventions in TIME magazine. His two TED Global talks have been watched more than five million times.
Keep up-to-date with Ingenia for free
SubscribeOther content from Ingenia
Quick read

- Environment & sustainability
- Opinion
A young engineer’s perspective on the good, the bad and the ugly of COP27

- Environment & sustainability
- Issue 95
How do we pay for net zero technologies?
Quick read

- Transport
- Mechanical
- How I got here
Electrifying trains and STEMAZING outreach

- Civil & structural
- Environment & sustainability
- Issue 95