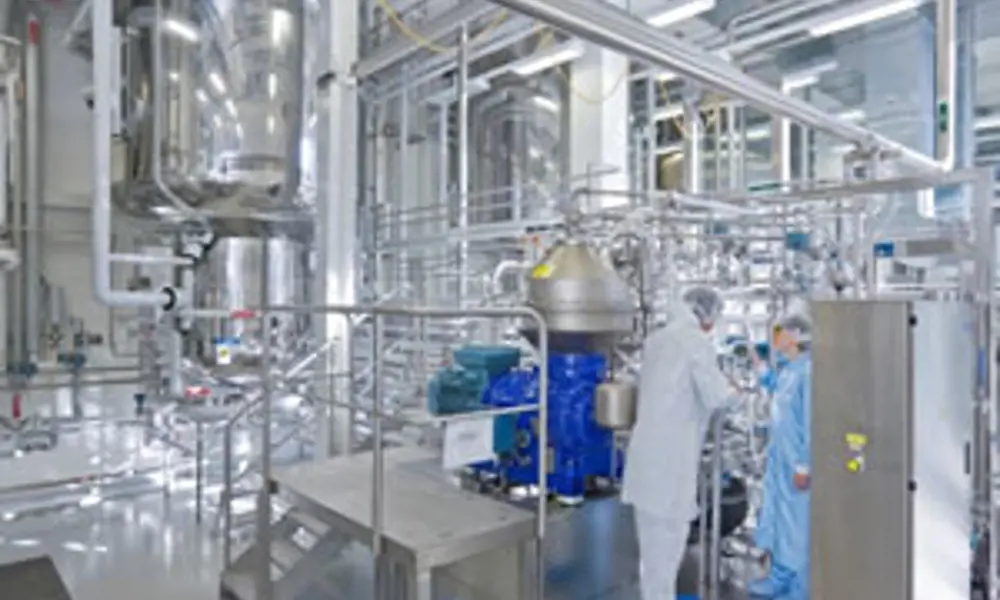
Biochemical Engineering
The large-scale centrifuge in the foreground separates the cells from the culture fluid. It is now possible to predict accurately the separation efficiency of a full-scale centrifuge using scale-down techniques employing just μL millilitres of volume © Lonza
MAKING MOLECULES FOR THE 21ST CENTURY MEDICINE
Medical researchers working on a variety of diseases are increasingly interested in tackling them through the use of a new generation of medicines that utilise the very specific biological actions of certain large molecules. Stephen Vranch FREng and Professor Nigel Titchener-Hooker FREng outline the significance of this development, and describe the challenges facing the biochemical engineer whose task it is to turn the discoveries into marketable products.
It was in the early 1900s that the German physician and scientist Paul Ehrlich discovered the anti-syphilitic action of the compound known as Salvarsan and so launched the era of modern chemotherapy. But medical research moves on. Hugely effective though they have been over the past century, the many drugs developed during this time have not generally mimicked the kind of molecules that the body itself uses to regulate most of what goes on within it.
From the hormones that influence growth to the antibodies that fight infection, the body employs very large molecules, many based on proteins, to achieve its goals. Doctors, by contrast, have relied mainly on the use of smaller, non-protein molecules to combat disease and correct physiological abnormalities. But more recent research findings make it apparent that to tackle some medical problems effectively, or even at all, doctors will have to intervene directly in the body’s own control systems. To acquire a more subtle, more targeted and so more effective influence, they too are turning to large molecules. Hence the need for what are loosely referred to as ‘biopharmaceuticals’: large molecule products of the biotechnology industry.
The first biopharmaceutical was synthetic human insulin, developed in the early 1980s using the technology of recombinant DNA: ‘genetic engineering’, to use the then-popular term. The rate of production of new molecules has since grown rapidly, and this is where a growing part of the future of drug therapy now seems to lie. The global market for biopharmaceuticals is large and expanding. In 2010, for example, it was worth $114 billion, with spending on R&D at over $25 billion; the same year saw 11 new products entering the market. In Europe in 2011, total pharmaceutical sales were €140 billion of which €36 billion was biopharmaceuticals.
The living workhorses of large molecule production were originally bacteria. But it has since proved possible to use cultured mammalian cells, including those of human origin, for making medically useful molecules. Structurally, these molecules may be indistinguishable from those we produce naturally, which makes them even more medically relevant. It is on the use of mammalian cells, with its difficulties as well as advantages, that this feature will focus – see Table 1.
The advent of biopharmaceuticals has stimulated the development of new skills in drug design and manufacture. The UK and Ireland lead in many aspects of bioprocessing, and employ hundreds of researchers as well as manufacturing staff. Key to this are the biochemical engineers whose training equips them to convert the potential of life science discoveries into practicable, economic and safe processes and products for the benefit of human health. The biochemical engineer is to these new medicines what the civil engineer is to a bridge, a road or a dam. The tools are different, as is the scale of the product. But the intention is identical: to design and make novel structures for predetermined purposes.
The products themselves include vaccines, blood clotting factors, clot dissolving agents, the hormone molecules that perform a signalling role in the control of all sorts of physiological processes, and monoclonal antibodies which attach themselves specifically to a single target in the body and can be used for diagnostic purposes or to deliver a toxic payload to a chosen site. Collectively, the new products are finding applications in therapeutic areas ranging from oncology and infection to neurology and diabetes.

Chinese hamster ovary (CHO) cells in a cell culture flask. CHOs are a cell line often used in biological and medical research and commercially in the production of therapeutic proteins © Alcibiades/Wikipedia
CELL FACTORIES
Each cell of the body is a tiny factory capable of making a huge variety of large protein molecules, some structural, some for catalysing chemical actions, some to act as local or remote messengers that determine the body’s physiological adaptation to changing circumstances or regulate the activity of its genes. Biochemical engineers borrow from nature; they utilise living things’ capacity for synthesising complex molecules. Where the medical need is simply for more of a naturally occurring molecule – a hormone, for example – cells can be set to work doing what they normally do. Where the need is for a variant of a natural molecule, or for a product that the cell does not normally make, it may require genetic modification.
A researcher choosing a cell for making a particular product will usually start with one of the established cell lines on which most laboratory research is carried out and which are recognised and approved by the pharmaceutical regulating agencies – see Table 2. Some of these cells were derived originally from animals, some from humans. Most celebrated of the latter is the HeLa cell line. Created in 1951, its name commemorates the American woman Henrietta Lacks from whose cervical cancer the cells were originally taken. Henrietta Lacks herself died later in the same year, but through the HeLa cell lines has achieved immortality.
Using the techniques of molecular biology, any genes that may be needed to instruct the cells of a particular line to make the required product are inserted, and a quantity of the newly modified cells is then grown up and prepared for testing and storage.
It is essential for any modified cell line to be fully characterised and shown to be free from harmful viruses that could have come from the source animal or human. It must also be shown to be both productive and stable through many generations. Before granting a manufacturing licence, drug regulatory bodies have to be convinced that the cells can be relied upon to go on making the product consistently for years. Once these hurdles have been crossed, the manufacturers will harvest a quantity of the cells, deposit them in a series of separate ampoules, and freeze them for storage. The genetic make-up of cell lines can change rapidly, even during the course of a period of cell culture lasting perhaps no more than a few hundred hours. But innovations in cell line development and genomics now allow the genes that make the biopharmaceutical proteins to be monitored as they function within the cells. The progress can be monitored as it happens.

A large bioreactor for growing mammalian cells. The production vessel is fed with sterile nutrients from the mezzanine level above it © Lonza
INTO PRODUCTION
To begin production, a single ampoule is thawed and its cells are allowed to recommence growing and multiplying, first to a laboratory scale of typically less than one litre, and then successively in containers of increasing volume up to the production scale of 20,000 litres or more. At this point, a typical cell concentration would be around five million cells per millilitre. Even after the passage of many generations cells will continue to make the required protein product.
The containers, or ‘bioreactors’, in which this growth takes place are thin-walled pressure vessels usually made of a highly resistant grade of stainless steel. The solution in which the cells are cultured was formerly based on animal-derived ingredients such as serum. This created the risk of viral contamination from the animal source. Nowadays, most culture media contain only synthetic components such as essential vitamins, inorganic salts, carbohydrates, lipids, and amino acids dissolved in pure water. The solution is sterilised by passing it through a filter medium with pores of less than 0.2µm diameter. Conventional bioreactors are fitted with piped flows not only of the required media solutions but of sterile air to provide the cells with oxygen. Impellers are used to provide vigorous mixing of the vessel’s contents. Although these contents may require initial warming to stimulate the cells’ metabolic activity, their own output of energy is then sufficient to keep the interior of the bioreactor at the typically required temperature of 35.0 ± 0.5°C.
The eventual scale-up from laboratory to commercial production is preceded by a scale-down exercise designed to ensure that the manufacturing plant proposed by the biochemical engineers will actually achieve the desired results. This is critical because the shear forces generated within the manufacturing equipment can easily damage large, complex molecules. New scaled-down tools have been created that allow the key design parameters to be assessed not by conventional geometric scaling rules, but by considering the actual forces with which a molecule will be faced during manufacture.
At the industrial scale, machines generating 10,000g of centrifugal force will be used to separate the various components of the mixture leaving the bioreactor. Any damage likely to occur can nowadays be predicted by testing tiny quantities of material in specially designed shear cells. Scale-down factors as high as 107- fold can be accommodated; accurate predictions of the effects of centrifugal operation at the 20,000L scale can be determined using as little as 10ml of material. Figure 1 shows a full-scale centrifuge in a production facility.
ISOLATION AND PURIFICATION
By the end of the growth and production phase, the amount of the protein in a cell culture fluid will typically range from 2 to 10g/litre. The challenge then is not only to concentrate it, but to separate the molecule from the other chemicals and cellular material in the ‘broth’. Purities in excess of 99.99% are required, and any contaminants present may have to kept below 1ng/dose. The challenge of achieving this has been likened to dropping broken eggs into a swimming pool and then extracting the egg white. Moreover, the separation must be achieved without damaging the proteins and so undermining their therapeutic activity. As already mentioned, the very size and complexity of these molecules renders them susceptible to the shear forces produced by pumps, valves and high-speed continuous centrifuges.
A knowledge of the physical and chemical properties of the molecule to be extracted will offer an indication of how best to separate it from the associated impurities. Where the product remains in the cells that produced it, these must be disrupted to release their contents into the culture medium. The protein product is then separated from cell debris and any remaining whole cells by filtration or centrifugation. Membrane filters with pores only a few nanometres in diameter can retain proteins while allowing impurities to pass through. Most animal cell lines will contain viruses; these too must be removed by appropriate filtration.
Purification and retrieval of just the protein of interest is usually by chromatography. Most protein molecules are electrically charged, which allows particular types to be removed using ion exchange chromatography. When passed through a column of beads carrying an opposite charge, a protein will bind selectively to the beads – from which it can subsequently be retrieved with an appropriate wash to produce a solution now rich in that protein. Different chromatographic columns, methods of chromatography, and washes can serve both to remove proteins from non-protein contaminants and to separate one protein from another, leaving just the desired product.
REGULATION
As with all medicines, the manufacture of biopharmaceuticals is heavily regulated. Products have to be safe, pure and effective; their manufacturing processes have to be reproducible, and validated to show that products have been made according to specification. Cleanliness and sterilisation are vital, so some companies favour disposable equipment, even for the bioreactor itself which may take the form of a pre-sterilised flexible bag. This has the additional benefit of increasing productivity by eliminating the downtime required for the bioreactor to be periodically cleaned and sterilised.
As any significant change to a manufacturing process is viewed as possibly affecting the product, re-testing is required to prove that the change has not modified the product’s clinical response in the patient. If new clinical trials are required, the cost could be more than £100 million and take more than a year to complete. Small wonder that the biochemical engineer specifying the process is under pressure to get it right the first time.
During most of its existence, the drug industry has manufactured its products in batches that are then subjected to laboratory analysis to verify their quality. Encouraged by regulators, and in particular the US Food and Drug Administration (FDA), more manufacturers are now using process analytical technology. This aims for quality by design in which the process effectively defines the product. It relies on a detailed scientific understanding of the chemical, mechanical and biopharmaceutical properties required of the product being manufactured. Indeed, it is not always possible to measure all the attributes of the product at the end of processing, and a complete knowledge of processing conditions is needed to ensure that the correct product has been made.
The quality of any biopharmaceutical is heavily dependent on the behaviour of the cell line responsible for generating it, and on processing conditions during purification. Cell lines need to be monitored to ensure that they are performing as expected. Pharmaceutical regulators mandate the use of systems and procedures that fulfil the requirements of current Good Manufacturing Practice (cGMP). The use of genetically modified organisms requires exceptionally high standards of engineering, design, operation and quality control. Manufacturers are regularly inspected to check their compliance with cGMP.
Biopharmaceuticals derived from cancer-like cell lines understandably attract special regulatory attention. The approval of tissue plasminogen activator (tPA) and erythropoietin (EPO) for the treatment of thrombosis and anaemia respectively proved to be regulatory milestones. The manufacturers of both these products had to demonstrate that, although derived from cancer-like cells, they posed no risk to the patient, and that the purification procedures had been successful in removing any potentially hazardous materials.
Facilities for the manufacture of biopharmaceuticals need to be designed and operated to high standards to ensure that, for example, the buildings used for making vaccines are not themselves the source of an infection, especially when the disease in question is not normally found in the region where the factory is located.
Regulators are often viewed in a negative light, and seen primarily as a force holding back new drugs from the market. In reality, while duly mindful of risks, regulators are not only experienced in what they do but often encourage innovation.

Figure 2. This figure shows a simulation of the adsorption of a molecule (lysozyme) as it is pumped though a bed packed with adsorbent particles. CFD techniques were used to predict the even flow of fluid through the bed which is critical for economy and the efficiency of purification
FUTURE CHALLENGES
Pharmaceutical manufacturing that relies on the close integration of biological and physical systems is hardly new. Microbial fermentation has long been one of the pharmaceutical industry’s standard technologies. But to create the large biopharmaceutical molecules described in this article is more demanding. Given that these molecules will play an increasing role in the practice of medicine in the future, it is vital that biochemical engineers anticipate the likely challenges, and consider how the manufacturing processes can be further improved. A fuller grasp of the principles that underpin all biochemical engineering, for example, will help to achieve more rapid scale-ups and so meet the pressure to move potential treatments more quickly from the laboratory bench and into commercial licensed manufacturing. The translation of good scientific ideas into products has long been a weak link in the development chain of new medicines in general.
There is also a need to design safe but more cost-effective processes for the manufacture of the new products that will eventually characterise the success of stem cell therapies in bringing about regenerative medicine. Flexibility may be a key virtue here; precisely what will be needed is not yet clear. With the cost of biopharmaceuticals being relatively high, there will be pressure to find innovative new technologies that can deliver the same quality and quantity, but at a lower price. Improvements to methods of continuous processing that boost yields, productivity and reproducibility will help here. So too will better containment of processes and equipment that avoid the need to conduct operations in expensive clean rooms. There may be more demand for systems that rely on single-use equipment.
The economy benefits greatly from the success of drug research and manufacturing. If biochemical engineers and life scientists can continue working together to meet the challenges posed by the need for effective biopharmaceuticals, the UK has the potential not only to maintain its current position in the sector, but to boost it.
Keep up-to-date with Ingenia for free
SubscribeOther content from Ingenia
Quick read

- Environment & sustainability
- Opinion
A young engineer’s perspective on the good, the bad and the ugly of COP27

- Environment & sustainability
- Issue 95
How do we pay for net zero technologies?
Quick read

- Transport
- Mechanical
- How I got here
Electrifying trains and STEMAZING outreach

- Civil & structural
- Environment & sustainability
- Issue 95